Liquid metals enabled advanced cryobiology: development and perspectives
Abstract
Cryosurgery and cryopreservation, as two important categories in cryobiology, have been impeded by the poor thermal conductivity of biological tissues or specimens. To improve this, diverse adjuvants, e.g., carbon-based materials, metallic nanoparticles, metallic oxide nanoparticles, etc., have been exploited to improve the heat transfer in heat-targeted regions to increase the tumor elimination efficiency as well as the post-thaw viability of cryopreserved specimens. Nevertheless, these materials suffer poor thermal conductivities, controversial biosafety problems, and high expense. Gallium and its alloys, as a class of room-temperature liquid metals (LMs), have been widely studied in the past decade for their low melting point, minor toxicity, outstanding transformability, and conductivity. Integrated with these superior properties, they have been widely applied in multiple fields, such as thermal management, flexible electronics, and soft robotics. Recently, our laboratory has been devoted to fusing LMs with cryobiology and has made a series of progress. In this article, we will first briefly introduce preparation pathways to LM-based functional nanomaterials and composites. Then, how these materials realize improvement in biological heat transfer will be presented, followed by a discussion about the biosafety of these materials, which is an essential concern for the cryobiological field. Recent studies employing LMs in advanced cryosurgery and cryopreservation will also be highlighted. The present challenges and prospects of LMs towards further development in cryobiology will be put forward to point out the possible research direction.
Keywords
INTRODUCTION
The field of cryobiology has made significant progress and has drawn increasing attention in the past decades since technologies derived from this category are closely related to the welfare of humankind, notably in areas such as organ transplantation, assisted reproduction, and tumor therapy. Despite encountering significant challenges throughout its development, the field has witnessed remarkable progress in recent years, largely attributed to the rapid advancements of materials science, which enable many exhilarating breakthroughs in cryobiology.
Cryosurgery is a medical technique that employs freezing to selectively eliminate undesired tissues (e.g., adipose, naevus, tumors, etc.) while minimizing cryogenic injuries to surrounding normal tissues. This technique has been promoted to replace a wide range of traditional therapies in tumor treatment due to its minor pain, fewer side effects, and minimal invasiveness to patients[1]. However, due to the intrinsic complex constructions and anisotropic thermal properties of living tissues, the frozen region is always unable to be precisely regulated to conform with irregular tumor tissues[2]. In some cases, freezing regions did not fully cover the tumor demarcation; thus, the low freezing efficiency failed to thoroughly kill the tumor cells. These technical limitations might lead to occasional recurrences afterward[1]; moreover, excessive freezing would ineluctably hurt adjacent healthy tissues and cause severe pain and side effects to patients. These defects were commonly ascribed to the ununiform and inefficient heat transfer within biological tissues. In order to overcome this, adjuvants were employed to enhance the freezing efficacy and regulate the freezing zone, thereby ensuring the elimination of tumors while minimizing damage to healthy tissues[3,4]. At the same time, these adjuvants could also: (1) Carry and deliver drug molecules to target tissues as assisted therapy; (2) Enable in vivo imaging for tracing and monitoring the progress of the therapy[5]. Currently, the well-studied adjuvants primarily consist of natural molecular adjuvants (such as antifreeze proteins[6,7], tumor necrosis factor alpha[8], and glycine[9]) and nanoparticles, for instance, MgO[10,11], Fe3O4[3,12], Al[13], and Au[14]. Among these, nanoparticles are capable of enhancing heat transfer, homogenizing temperature distribution, and, more importantly, regulating ice nucleation. However, rigid nanoparticles could poorly match soft biological tissues, potentially leading to abnormal cellular proliferation or cytokine secretion[15]. Thus, there is an urgent need for a soft and conformal material that can interact with tissues in a gentler manner and provide thermal enhancement.
Cryopreservation holds an opposite aim to cryosurgery instead of utilizing cryogenic media to cause damage; it intends to conserve biological structures and is regarded as the most promising approach to eternal preservation of biological resources since low temperature leads to metabolic inertness. However, this technique is also confronted with a huge challenge to preserve vulnerable cells or tissues and large-volume organs or even living organisms[16]. The conventional slow-freezing method causes inevitable ice formation in biospecimens and prolonged exposure to toxic cryoprotectants (CPAs), leading to potential abnormalities in cell phenotypes, attachment, gene expression, and differentiation[17]. Thus, slow-freezing methods could hardly be applied to large-volume tissues and organs[16]. The emergence of the vitrification technique seems to bring a silver lining. Vitrification aims to deeply cool down biospecimens without any bulky ice formation during both the cooling and warming processes[16,18,19]. Therefore, vitrification is considered as a prospective strategy to tackle subsistent challenges and make a breakthrough in the long-term preservation of complex tissues and large organs[16]. Vitrification aims to transit the liquid system into an amorphous glass state instead of a crystalline state, thus avoiding the mechanical destruction of cellular structures caused by ice formation. The vitrification process can be comprehended as a competition between system cooling and crystallization. Taking a homogeneous aqueous system as an example, when the temperature continuously drops below its melting point, the system will be in a supercooled state, and ice crystallization tends to happen. Yet, crystallization, consisting of ice nucleation and growth, requires a certain time. Also, the ice nucleation rate and growth rate highly depend on the degree of supercooling. There is a most promotive temperature for these two processes, respectively. Moreover, As the temperature drops, the dynamic viscosity of the solution will increase, and the diffusion coefficient will, thus, be decreased, which is unfavorable to ice growth[20]. When the temperature drops below a certain point, neither of these two processes is favorable, rendering the system incapable of crystallization[21]. Hence, as long as the cooling rate of this system is fast enough so that its temperature drops below its glass transition temperature (Tg), no ice crystal will form in this system, and it will turn into a glass state. The Tg is also believed to increase with a higher cooling rate[22], which means the system can more easily transit into a glass state with a higher cooling rate. Therefore, it is comprehensible that high-concentration CPAs are always essential to help realize vitrification: (1) They could decrease the melting point and increase the Tg of the system, making it easier to pass through the temperature region that is favorable for ice nucleation and growth; (2) High-concentration CPAs could increase the viscosity of the system, hindering the molecular locomotion from the solution to the surface of ice nuclei.
From this perspective, it is comprehensible that high-concentration CPAs are essential to increase viscosity and suppress ice nucleation and growth. However, the resuscitation of vitrified biospecimens is rather challenging than cooling because the ice nuclei forming during the cooling process tend to expand and burst during rewarming processes. Cooperatively, the cooling and warming processes both need to be rapid enough to ensure the phase change to overcome ice formation. Usually, for a certain CPA recipe, the critical warming rate (CWR) is several times higher than the critical cooling rate (CCR)[23]. The gold-standard water bath rewarming could hardly meet the criteria. Therefore, the idea of utilizing specific stimuli-responsive material-mediated heating effects to heat up cryopreserved biospecimens was proposed. Manuchehrabadi
Liquid metals (LMs) with low melting points have been drawing increasing interest in the past decades. Mercury is commonly used in routine life to be applied in thermometers, and its alloys and compounds have also been developed as dental fillers, cosmetic additives, and medicines[27]. Alkalis alloys also have subzero melting points and have been applied as a heating source in hyperthermia therapy for tumors[28,29]. However, these chemically unstable, hazardous substances require extra elaborative storage and operation, which restricts the application scope in the biological field. Recently, several studies have spotlighted gallium (Ga)-based LMs with superior chemical stability, low vapor pressure, and great biocompatibility[30]. The laboratory of the authors has been focused on studying fundamental behavior, characteristics, and applications in multiple fields. Based on previous research on cryobiology, our laboratory has been exploring the possibility of employing LMs in cryobiology to realize better outcomes. Thus, this review will only give attention to Ga-based LMs.
LMs feature inherently high thermal conductivity and flexibility simultaneously. They have been widely exploited as effective thermal interface materials (TIMs) to enhance interfacial heat transfer[31-33]. Through simple manipulations under room temperature and ambient pressure, LMs can be prepared into multiform combinational materials, including micro-/nano-scale LM particles with various morphologies[34]. Recently, it has also been proven that LMs are excellent metal solvents for nanoengineering or for producing various morphological metals[35,36]. The transformability of LM micro-/nano-particles (LMMPs/LMNPs) enables them to be applied in multiple fields, including printed electronics, drug delivery, biological sensing, etc. Moreover, resembling noble metal nanoparticles, LMNPs are also capable of displaying localized surface plasmon resonance (LSPR)[37], endowing them with high-efficiency PT conversion capability. As verified by a few works, LMNPs showed a considerable PT effect[38], consequently facilitating efficient elimination of cancer cells and inhibiting the regrowth of tumors[39-43]. Except for transforming into nanoparticles independently, LMs could also accommodate a wide range of micro-/nano-particles to form LM composites with modifications of specific characteristics[44]. In this perspective, the basic properties of LMs will be briefly introduced; interfacial issues are involved in almost all the manipulation of LMs, and they will be emphatically discussed. Then, based on these impressive properties and phenomena of LMs, their recent advanced applications in cryobiology will be presented [Figure 1]; lastly, from the perspective of future development of LMs in cryobiology, possible future application scope will be proposed.
LM-BASED FUNCTIONAL MATERIALS
Preparation of micro-/nano-LM
The liquid nature of LMs enables them to be easily prepared into various modalities. Compared to rigid metallic nanoparticles, which are practically synthesized by reductive methods, LMs could be scaled down through a facile top-down strategy. To obtain LMMPs or LMNPs, the probe-sonication method is usually applied. Generally, bulk LMs are placed in a dispersant solution (e.g., deionized water, ethyl alcohol), and then, the ultrasonic probe induces cavitation to smash LMs into smaller particles with oxide skin covered. During the sonication process, small particles still have the possibility to collide with each other and coalesce together to form larger particles. Several factors, such as activation power, sonication time, and temperature, can influence the sonication efficiency and the size of particles. At the beginning of sonication, the mean diameter decreases as time extends, and some large bulks remain unbroken in this state. As sonication keeps acting, the mean diameter becomes smaller till a minimum value. The mean size of LMNPs is highly correlated to the LM type, solvent properties, temperature, mass ratio, etc. The sonication power has no impact on the eventual size of LMNPs but only affects the time spent in reaching the eventual state[45,46]. High temperature tends to result in a larger particle size, which is probably attributed to the decrease of the cavitation effect, reducing the break-up ability of sonication[46]. In practice, for the purpose of acquiring relatively uniform and small LMNPs, static or centrifugal precipitation of large particles is always essential[47,48] [Figure 2A].
Figure 2. (A) Preparation of micro-/nano-LMs through sonication. (a) Core-shell structure of LM particles; (b) The influential factors of the LM particle size after sonication; (B) LMs incorporated with metal particles and the main mechanisms. Mechanism 1: Metal particle internalization through sticky LM oxide; Mechanism 2: Metal particle internalization through the formation of metallic bonds; (C) Transformability of LMMPs/LMNPs. (a) Influential factors on the structure of LM particles; (b) Cold-induced transformation and the change of the interfaces between LMs and water. LMs: Liquid metals; LMMPs: LM micro-particles; LMNPs: LM nano-particles.
LMs incorporated with other micro-/nano-particles
Despite the inherent merits of LMs, researchers still sought to further improve their performance in multiple aspects. Fortunately, the liquid nature provides LMs a universal platform to combine with diverse materials through simple mechanical agitation methods. Chang et al. revealed the underlying mechanism of this process. While agitating the mixture of LM and nanoparticles, air-induced gallium oxide skin is continuously cracked into pieces, and new oxides occur synchronously. Nanoparticles can hardly enter the LM because of the intrinsic high surface tension. Nevertheless, they become ensconced in adhesive oxide debris while being agitated, enabling easy internalization of the nanoparticles by LM[49]. As a result, LMs successfully integrated diverse functional nanoparticles [Figure 2B].
Ga-based LMs could also incorporate with metallic nanoparticles through intermetallic wetting, which is induced by the formation of strong metallic bonds. Tang et al. disclosed the intermetallic wetting-induced LM phagocytosis of copper nanoparticles[50]. Based on the experimental phenomena of biomimetic phagocytosis behavior of LM droplets and the theoretical calculation of surface free energy, they conclude that: (1) The autonomous internalization behavior is a complete wetting phenomenon; (2) Irreversible intermetallic wetting provides sufficient force to overcome the energy barrier required for the transition from non-wetting to wetting state. In another work conducted by Tang et al., a typical intermetallic phase, CuGa2, was observed and characterized to exist stably in GaIn-Cu composites[51]. Doping with copper microparticles led to an impressive alteration in mechanical properties, thermal and electrical conductivities, and melting/solidification points. In this manner, the characters of LM composites could be regulated more precisely compared to the agitation approach. Adopting this technique, GaIn-Cu, with improved thermal and electrical conductivity, has been applied to flexible electronics[52] and enhanced cryoablation[53].
Following the voltage-facilitated Cu particles doping approach proposed by Tang et al., Hou et al. prepared GaIn-Cu composites to enhance the cryoablation efficacy[51,53]. This principle also provided inspiration for Park et al. to introduce LM-lyophobic carbon nanotubes (CNTs) to be finely incorporated with eutectic gallium-indium (EGaIn), where Pt was selected as an intermediary agent[54]. In summary, the examples of metal particles@LMs are listed in Table 1.
Examples of LMs incorporated with micro-/nano-particles
Liquid metal composition | Doped particles | Approach (reagent) | Doping content (wt%) | Improved properties | Ref. |
GaIn24.5 | Ni MPs | Mechanical stirring | 3%-9% | Adhesion | [49] |
GaIn24.5 | Cu MPs | Voltage facilitation (NaOHaq) | 5%-17% | Thermal, electrical conductivity & shapeability | [51] |
GaIn24.5 | Cu NPs | Mechanical stirring | 5%-20% | Viscosity | [52] |
GaIn24.5 | Cu MPs | Voltage facilitation (NaOHaq) | 29% | Thermal conductivity & shapeability | [53] |
GaIn24.5 | Pt-CNTs | Mechanical stirring; NMP | 3%-15% | Thermal and electrical conductivity | [54] |
GaIn24.5 | Quartz MPs | Mechanical stirring or ball milling | UTD | Printability and recoverability | [55] |
GaIn24.5 | Mg MPs | Mechanical stirring | 0.5%-3% | PT conversion efficiency and shapeability | [56] |
GaIn24.5 | Fe NPs | HClaq | UTD | Magnetization | [57] |
Ga67In20.5Sn12.5 | Fe MPs | HClaq | 2%-20% | Elongation | [58] |
Ga68.5In21.5Sn10 | W MPs | Oxide wetting | 10%-90% | Enhanced thermal conductivity | [59] |
GaIn24.5 | Fe NPs + MXene | Self-assembly of MXene | UTD | Shape deformability and locomotion | [60] |
Transformability of LMMPs/LMNPs
Due to the high surface tension, the oxide-covered LMMPs/LMNPs are typically spherical. However, under certain conditions, LM particles can be shifted into diverse shapes. These anisotropic nanoparticles possess specific optical, electrical, and thermal properties[61]. The shape transformation of LM particles could be realized by multiple means. Lin et al. sonicated EGaIn in deionized water containing positively charged surfactants and obtained LM nanospheres. Then, they heated the sample at 70 °C for 30 min, and the nanospheres transformed into nanorods. They further manifested that such a morphology shift was due to the production of gallium hydroxide (GaOOH)[62]. Gan et al. prepared polydopamine-coated LMNPs and took advantage of the PT conversion capability of polydopamine to heat inner LMs, triggering shape morphing of LMNPs from spheres to ellipsoids[48]. Li et al. performed sonication with relatively high power (800 W) and extended duration (120 min) to maximize oxidization and obtained LM nanorods[63]. Sun et al. sonicated LMs in an aqueous solution containing cetrimonium bromide, a positively charged surfactant, to obtain gallium and EGaIn nanorods[40]. In addition to sonication methods, due to the liquid nature of gallium, Wang et al. adopted a pressure-derived filtering approach to fabricating homogeneous gallium nanorods at 35 °C[64]. As reported by Sun et al., different morphologies of LMNPs will result in varying thermal properties (e.g., thermal conductivity due to the composition change, specific absorption rate, PT efficiency, etc.) and biodegradability[40].
Sun et al. reported an intriguing transformation behavior of LMMPs under a space-restricted two-phase condition[65]. When the LMMPs were cooled in a solution with a higher melting point, the basal solution would first solidify and trap the LMMPs. Then, as the temperature continued to decrease, the LMMPs would expand due to the abnormal volume expansion phenomenon and explode to form spikes to pierce the surrounding ice. This phenomenon offered a distinct inspiration to exert excess mechanical damage to tumor tissues in cryosurgery [Figure 2C].
THERMAL PROPERTIES OF LM
With the development of cryobiological techniques, precise and rapid temperature control is essential to achieve optimistic outcomes. However, it is hindered by the intrinsic low thermal conductivity of biomaterials[66]. Inferior heat transfer results in failures in both cryoablation and cryopreservation, e.g., insufficient elimination of cancer cells, damage to healthy tissues, ice recrystallization during thawing of the vitrified biosamples, etc. To tackle this challenge, scientists have added adjuvants to improve the heat transfer and homogenize heat distribution. Ideally, nanomaterials with self-heating effects (e.g., GNRs,
Thermal properties of Ga-based LMs and common substances
Material | Melting points (°C) | Thermal conductivity (W·m-1·K-1) |
Water | 0.0 | 0.599 |
Ga | 29.8 | 29.4* |
GaIn20 | 16.0 | 26.58 |
GaIn24.5 | 15.5 | 26.58 |
Ga67In20Sn12.5 | 10.5 | 39 |
Hg | -38.9 | 8.34 |
Au | 1,064.2 | 317 |
Fe | 1,538.0 | 80 |
Cu | 1,083.4 | 401 |
Skin | - | 0.29※ |
Muscle | - | 0.60※ |
Blood | - | 0.48※ |
Enhanced heat transfer
The inferior thermal conductivity of biomaterials is an inevitable barrier to the regulation of their temperature with rapid response, wide range, and high precision. Over the past two decades, our laboratory has extensively studied the LMs as coolants[67-70] or TIMs[31-33,71], which has confirmed their ability to enhance heat transfer and diminish contact thermal resistance [Figure 3A]. For biomedical applications, Wang et al. prepared EGaIn-based composite pastes to actualize thermal therapy for subcutaneous tumors[56,72]. The modified LMs were tightly adhesive to skins and increased the heat penetration into tumor tissues according to the simulation results. Hou et al. testified to the effectiveness of the probe cryoablation and manifested the increased “cold energy” transfer enabled by conformally coated LM paste in both numerical simulation and experiments[53].
Figure 3. (A) Remarkable interaction between LMs and biomaterials. (a) Soft interaction between LMs and biomaterials without any stiff damage; (b) Conformal adhesion between LMs and biomaterials; (B) The ability of LMs to enhance the heat transfer in different modalities under various conditions; (C) Superior stimuli-responsive properties of LMs over commercial nanomaterials; (D) Synergistic treatment of combining thermal property of LMs with chemotherapy and embolization. LMs: Liquid metals; LMNPs: LM nano-particles; PT: photothermal.
In addition to the bulk LM-enabled heat transfer improvement, the idea of “nano-cryosurgery” has been proposed, whereby solutions containing nanoparticles (i.e., nanofluids) are injected into the tumor site to enhance heat transfer, aggravate freeze damage, and regulate ice ball formation. For a solution containing nanoparticles, its thermal conductivity can be estimated by Maxwell-Garnett’s model[73]:
where keff, kbase, and knp are the thermal conductivity of nanoparticle-dispersed solutions, basal solutions, and dispersed nanoparticles, respectively, and Ø is the volume fraction of nanoparticles. According to this model, we can speculate that with higher concentration and higher intrinsic thermal conductivity of nanoparticles loaded, the thermal conductivity was significantly increased. Di et al. implemented nanoparticles-mediated cryosurgery and figured out that MgO nanoparticles could significantly increase the thermal conductivity of tissue and promote the ice ball formation. The histological characteristic of the frozen area also indicated that MgO enhanced cryosurgery to biological tissues[10]. Hence, surgeons can regulate the ice formation in spatial and time domains by injecting nanoparticles at specific concentrations and into certain regions[1].
Stimuli-responsive heating effect of LMs
For vitrified cryopreservation, sufficiently rapid and uniform heating of the biospecimens is key to the success of vitrification. In addition, a high concentration of CPA with a low critical cooling/warming rate is required. To meet the criteria, containers have been designed with small volumes and large contact surfaces to achieve high cooling/warming rates. However, it would largely restrict the practical application of vitrification techniques. To tackle this challenge, the scientists added self-heating adjuvants to the CPA recipes to accelerate the thawing of the biospecimens. Several nanomaterials have been manifested as PT (e.g., GNRs[25], MoS2 nanosheets[74], MXene nanosheets[75], etc.) or magneto-inductive agents (e.g., iron oxides[24,76,77], metallic foams, foils, and meshes[26]) to successfully thaw vitrified biospecimens. This technique has many advantages over conventional convective rewarming methods: (i) non-contact; (ii) tunable and extremely high heating rates; (iii) uniform temperature distribution; (iv) biospecimen scaling-up; (v) potential in reducing the dose of CPA, etc. Studies in recent years have demonstrated LMs with the capability of self-heating in both modes, which will be presented in this section, as shown in Figure 3B.
Photothermal effect
As reported by several studies in recent years, the PT effect of LMNP suspension has been manifested. Chechetka et al. revealed the profound PT effect of LMNPs and verified its efficient damage to cancer cells[47]. They claimed the PT conversion efficiency of as high as 52%, which is much higher than commercially used AuNR1 (17%) [Figure 3C]. The spectroscopic evidence showed no absorbance peak in vis-near-infrared ray (NIR) regions. It means that the underlying mechanism of PT effects of LMNPs may be distinguished from that of gold nanoparticles, which is known to be due to LSPR[78]. So far, the underlying mechanism of the PT effect of LMNPs has not been revealed explicitly. Nonetheless, this effect has still been widely studied and extended to realize diverse functionalities. Sun et al. prepared variform LMNPs and characterized their morphology-dependent PT conversion efficiency[40]. The stability of PT agents is always of great concern, especially considering the fact that temperature rise causes oxidization and morphing of LMNPs, as discussed earlier in this paper, which would cause exacerbation of the efficiency. Wrapped with non-PT coatings, LMNPs were able to resist shape morphing and realize stable repeated self-heating. Hu et al. manifested that LMNPs coated with mesoporous silica displayed enhanced immobilization and sustained PT effect[79]. Besides direct hyperthermic damage to cancer cells, the PT effect could also cooperate with chemotherapy or embolization to realize synergistic treatment [Figure 3D]. Grafted with functional ligands, LMNPs could carry anti-tumor drugs and release them when triggered by self-heating under irradiation, which further improves the tumor elimination efficiency[47,79,80]. Wang et al. integrated PT treatment, chemotherapy, and embolization in one combinational material[57]. They successfully encapsulated EGaIn-Fe nanoparticles and doxorubicin hydrochloride into alginate hydrogels. This hybrid agent could be immobilized at the main artery of the tumor, perform the PT effect under irradiation, and thus trigger drug release to the targeted tumor tissues.
Inductive heating
In recent years, magnetic inductive heating has been studied in tumor therapy and vitrified cryopreservation. Compared to PT techniques, the actuation range is restricted by irradiation area, and magnetic inductive heating holds two major superiorities: (i) a larger actuation scale and (ii) better flexibility of manipulation. Fe3O4 is the most applied magnetic agent for inductive heating. Manuchehrabadi et al. applied Fe3O4 nanoparticles and manifested the capability of magneto-inductive heating to rapidly and uniformly thaw vitrified arteries up to 50 mL[24]. Zhan et al. applied this technique with carboxylic acid-modified Fe3O4 to resuscitate cryopreserved whole rat kidneys and achieve integral structures[76]. Han et al. applied this strategy to vitrify rat kidneys for 100 days and rewarmed them with a nanowarming strategy. They transplanted post-thawed kidneys into receptor rats and found that the renal functions recovered back to normal levels after 2-3 weeks after early dysfunction[81]. As metallic materials, LMs are also appropriate for this technique. Under an alternating magnetic field, an eddy current will be produced due to Faraday’s law of induction, thus generating heat inside the metal. Wang et al. demonstrated a more considerable heat generation of LM than Fe3O4 in an alternating magnetic field[82] [Figure 3C]. Then, by modifying LMs with polyethylene glycol (PEG) and doxorubicin (DOX), they realized thermo-chemo hybrid therapy. In another work, Wang et al. prepared oxidized EGaIn to conformally coat the skin of mice and generate considerable heat under an alternating magnetic field to actualize thermal therapy for subcutaneous tumors[72]. With such a highly efficient inductive heating effect, LMs hold great potential in vitreous cryopreservation.
BIOSAFETY
In biological applications, the safety of materials always stands as the top priority. Unlike mercury, which easily vaporizes in the atmosphere and causes inhalation risk, Ga-based room-temperature LMs have extremely low saturated vapor pressure, thereby eliminating the need for special packaging for long-term storage. Despite the natural stability, the toxicity of LMs in biological environments still attracts great attention. Both in vitro and in vivo toxicity of LMs, whether in bulk formation or particles, will be discussed in this section.
Ex vivo cytotoxicity
For Ga-based LMs, the major factor that causes cellular toxicity is ionic release. Kim et al. systematically evaluated the ionic toxicity of EGaIn in the aqueous environment[83]. For bulk EGaIn, Ga3+ concentration increased with soaking time until saturated, while In3+ stayed at a negligible level. The cytotoxicity was evaluated by pre-soaking LMs within the growth media for 24 h, followed by co-culturing cells with these media for different days and viability characterization. Their results showed that bulk EGaIn had little impact on the cell viability and proliferation. However, when LMs were sonicated to micro-/nano-particles, the concentration of In3+ was increased more than 1,000 times within 20 min of sonication [Figure 4A]. This was probably ascribed to the increased surface area-to-volume ratio, which resulted in increased interfacial interaction between LMs and solutions. This process also allowed more In to appear at the surface of LM particles and interact with the solution. In vitro assays indicated significant damage to cells caused by releases from LM nanoparticles (with a mean size of less than 500 nm) within only one day of co-culture [Figure 4B]. Given the fact that ultrasonication caused a significant change in In3+ rather than Ga3+ and led to a decrease in cell metabolic activity, it could be speculated that In3+ gave rise to more critical cytotoxicity than Ga3+.
Figure 4. (A) In3+ concentration with extended sonication time. Reproduced with permission[83] Copyright 2018, American Chemical Society; (B) Live/dead staining of HeLa cells incubated with media containing releasates. Green and red dots indicate live and red cells, respectively. Reproduced with permission[83] Copyright 2018, American Chemical Society; (C) Cytotoxicity of alginate hydrogel encapsulated LMNPs to three different cell lines. Reproduced with permission[57] Copyright 2021, the Royal Society of Chemistry; (D-F) Biocompatibility of LM-based composites in tissue levels: No significant histological damage was found in main organs (D) Reproduced with permission[40] Copyright 2019, the Royal Society of Chemistry; Indicators of hepatic (E) and renal (F) functionalities after in vivo injection, Reproduced with permission[72] Copyright 2019, the WILEY-VCH Verlag GmbH & Co. KGaA, Weinheim; (G) Body weight of mice after in vivo injection. Reproduced with permission[57] Copyright 2021, the Royal Society of Chemistry. LM: Liquid metal; LMNPs: LM nano-particles; TVI: tail vein.
In recent years, there has been much research that adopted LMs in biomedical applications and studied their biosafety in different hierarchies. At the cellular level, their results are generally in accordance with the above discussion. Large-scale LMs tend to show no significant damage to various cell lines[72,84,85]. Conversely, LM nanoparticles showed more or less damage to cells; e.g., nanospheres prepared by Hou et al. and nanorices prepared by Yan et al. both exhibited inhibition to cellular metabolic capability[86,87]. However, it is noteworthy that Hou et al. only took a small amount of suspension after sonication to operate lyophilization and later re-dispersed LMNPs in cell culture media, which resulted in the dilution effect to relieve their in vitro toxicities[87]. As conjectured by Kim et al., the sonication process generated extremely localized heat and pressure and induced chemical reactions, which might encourage ion release[83]. Then, we can regard the eventual ion number of LM nanoparticles suspension as two parts: (i) the ion number right at the timepoint that sonication terminated (nson) and (ii) the number of ions released during the static standing of the post-sonication suspension (nsta). According to the result of Kim et al., we can speculate that nson >> nsta, indicating that dilution of primary dissolvents could probably remit the ionic toxicity[83]. To further tackle the ion-release problem, biocompatible encapsulation was applied to restrain the release of ions; e.g., Wang et al. prepared calcium alginate hydrogel packaged magnetic LM nanoparticles and verified their negligible toxicity to different cell lines with the concentration from 2.5 to 200 mg/mL[57] [Figure 4C]. For in vivo injection applications, the impact of LMNPs on the immune system of the human body is a critical issue. Zhang et al. studied ex vivo engulfing behavior of macrophages to different inorganic particles[88]. They observed that whether as individual particles or aggregated particle clusters, Ga NPs and other studied particles (Silica, Sn, In, Bi, and Au) could all be engulfed by macrophages. In terms of the cellular viability of macrophages incubated with these particles, Ga showed little cytotoxicity in 12 h with a safe threshold of 244.2 μg·mL-1. Generally speaking, Ga showed better cellular compatibility than In and Bi but is more toxic than Au and Sn. The authors also reported that particles with higher density cause a more pronounced decrease in the migration ability of macrophages. Among these particles, the density of Ga particles is only larger than silica and smaller than other metallic materials, and they were found to have a milder impact on macrophage movement. This work indicates that injection of particles might cause immunoreaction at cellular levels. Extensive work should be further carried out to verify their impact on the immune system in living bodies.
In vivo biocompatibility
Although the cellular toxicity of bulk LMs (and encapsulated LM particles) is evidently proved to be negligible, the in vivo biosafety is still of great concern. Cogitation includes inflammatory reaction, mechanical damages, the metastasis of materials and concentration effect, organ toxicity and hematic toxicity, etc.
A few works also systematically evaluated the influence of the in vivo introduction of LMs. Yang et al. operated a subcutaneous injection of 20 µL EGaIn and made a biopsy of vicinal tissues[89]. The H&E staining images did not show any obvious morphological alteration in the skin or muscles near the injection site, and no inflammation or muscle degeneration was found [Figure 4D]. In addition, the injected LM showed remarkable stability and barely metastasized to the main organs. Except for subcutaneous injection, Wang
ADVANCED APPLICATIONS OF LM IN CRYOBIOLOGY
Integrated with all the properties discussed above, LMs have the potential to be used in two opposite directions. Herein, we will show that LMs can either serve as ice inhibitors to protect biosamples during cryopreservation or exert enhanced damage to tumor cells in cryotherapy, determined by specific manipulation. Our laboratory has been striving to push forward the application of LMs in cryobiological applications and has made some significant progress in recent years, which will be presented in this section.
LM-mediated vitrification of cell suspensions
With the aim of no ice formation through the whole procedure, vitrification has been more widely investigated and holds the potential of increasing cell viability and scaling up the size of cryopreserved specimens[19,23,24,74,77]. On account of the acknowledged fact that the rewarming process is more challenging than the cooling process, high rewarming rates are crucial to successful vitrification. Inspired by the significant PT conversion effect of LMNPs, Hou et al. applied them as nanoscale heat sources that realized uniform and rapid rewarming of vitrified cell suspensions [Figure 5A i][87]. Moreover, the cell suspensions loaded with LMNPs showed mitigated devitrification compared with the control group [Figure 5A ii]. They utilized ultrasonication to prepare LMNPs, followed by the centrifugal screening of the particles and lyophilization of the supernatant, which ensured a uniform size distribution of the LMNPs. The in vitro biocompatibility test was consistent with the work presented above. The calculated PT conversion efficiency was 52%, which was higher than long-studied gold nanomaterials and in accordance with the previously reported value[47]. This consistency probably also suggested that surface modification might have little influence on the PT efficiency of LMNPs. Benefiting from the “nanowarming” technique of LMNPs, the CPA recipe they applied was free of dimethyl sulfoxide (DMSO), which is widely considered to be toxic to cells[90]; at the same time, the total CPA concentration was far lower than commercial recipes[23]. With a minimum dose of LMNPs (0.1 mg/mL), the viability of rewarmed cells exceeded 70%. Even in the experimental groups without laser irradiation, the cell viability was still uplifted with a higher dose of LMNPs [Figure 5A iii], probably ascribed to heat transfer enhancement of the suspension. The resuscitated stem cells were evaluated in multiple dimensions, including attachment efficiency, proliferation, expression of critical antigens and genes, and multi-directional differentiation ability. To demonstrate the potential of this technique in large-scale specimen cryopreservation, they further vitrified and rewarmed murine tails, resulting in better protection efficacy from the point of view of histological morphology analysis.
Figure 5. Advanced cryobiological applications of LM-based materials. (A) LMNP-mediated ultrarapid rewarming of vitrified biospecimens. Reproduced with permission[87] Copyright 2019, Acta Materialia Inc. (i) Illustration of vitrification of cell suspensions loaded with LMNPs and NIR laser-induced rewarming; (ii) Illustration of LMNPs inhibiting ice formation; (iii) Live/dead staining of resuscitated cells with/without LMNPs; (B) LM-mediated combined cryoablation and PTT. Reproduced with permission[53] Copyright 2020, American Chemical Society. (i) Illustration of LM paste coating enhanced cryoablation. Inset is the simulated result of temperature distribution with (left) or without (right) LM paste; (ii) Illustration of LMNP-mediated PTT. Inset is the in vivo infrared thermographic image of radiated tumor tissues bearing LMNPs (left) or not (right); (iii) Post-treatment cellular viability; (iv) Change of post-treatment tumor volumes; (C) Freezing-induced LMMP deformation enhanced cryoablation. (i) Illustration of probe-cryoablation and LMMPs deformation enabled mechanical damage to tumors. Reproduced with permission[85] Copyright 2020, Wiley-VCH GmbH; (ii) Deformation ratio of LMNPs in different solutions. Reproduced with permission[65] Copyright 2020, American Chemical Society; (iii) Post-cryoablation tumor volume under different conditions. Reproduced with permission[85] Copyright 2020, Wiley-VCH GmbH; (D) Liquid metal transformer enabled endosomal escape for enhanced cryo-treatment. Reproduced with permission[91] 2021 Elsevier Inc. (i) Illustration of cell membrane-wrapped gallium particles entering tumor cells; (ii) Deformation of gallium particles causing damage to endosomes, facilitating endosomal escape; (iii) Tumor volume after cryoablation with different conditions. DMSO: Dimethyl sulfoxide; LMMPs: LM micro-particles; LMNPs: LM nano-particles; NIR: near-infrared ray; PTT: PT therapy.
This work primarily exploited LMNPs as functional materials in cryopreservation protocol, which mainly made differences from three aspects: (i) No DMSO was added to the CPA recipe; (ii) Dispersing uniformly in CPA to increase the thermal conductivity of suspension; (iii) Serving as PT sensitizers to rapidly rewarm the vitrified specimens to avoid recrystallization. Combining their thermal properties, modifiability, softness, and biocompatibility, LMs hold great prospects in the field of cryopreservation.
LM-enhanced tumor thermotherapy
LM-mediated ice-fire ablation
Combining high thermal conductivity, PT effect, and conformability, Hou et al. proposed an LM-based hybrid platform to improve the efficacy of tumor therapy[53], which comprised two major parts: (i) They prepared pasty LM-Cu composites to apply a conformal coating on the skin to enhance the heat transfer. As shown in Figure 5B i, the simulation result indicated that LM paste coating could result in deeper penetration of cold energy into the tumor. They injected LMNPs into the tumor and radiated the tumor with NIR to implement PT therapy (PTT) [Figure 5B ii]. The near-infrared thermograph demonstrated the phenomenal PTT effect aroused by LMNPs. Furthermore, to further exploit the therapeutic effect of the LM platform, Hou et al. combined these two modes successively[53]. The tumor cells underwent drastic temperature change from extreme hypothermia to hyperthermia, which would also cause intensive thermal stress to ultimately kill tumor cells [Figure 5B iii]. The experimental result manifested that LM platform-mediated cryoablation combined with PTT realized better tumor elimination efficiency than each therapy alone [Figure 5B iv]. Besides, only in the combined therapy group the tumor did not recur.
LM deformation enhanced destruction of tumors
Sun et al. discovered an intriguing deformation behavior of LMMPs in a dual-liquid phase system. However, although the melting point of gallium is 29.8 °C, due to the size-dependent supercooling effect, Sun et al. characterized the phase transition temperature to be between -20 and -60 °C, which is much lower than the chitosan solution[75,85]. Therefore, when the temperature decreased, the chitosan solution froze first, and LMMPs were trapped within ice. When LMMPs underwent phase change, they would expand and form spiny deformations to cause mechanical damage to the surrounding ice within only 1 ms[85], as shown in Figure 5C i. The deformation ratio of LMMPs is determined by the properties of the surrounding solution
In order to increase the targeting ability and increase the destructive efficacy, Wang et al. assembled cell membrane-encapsulated Ga particles (Ga/MPs) with the ability to be delivered into cancer cells to exert cactus-like deformation inside endosomes under freezing to achieve endosomal escape[91] [Figure 5D i and ii]. They demonstrated the prominent targeting capability of Ga/MPs through fluorescent staining tracing methods. The fluorescence microscopy images show co-localization of endosomes and Ga/MPs. After freezing treatment, the co-localization area significantly decreased, indicating more endosomal escape caused by Ga/MPs. With the injection of Ga/MPs, the cryosurgery efficiently inhibited tumor growth compared with the outcome of the group without cryosurgery. In addition, without membrane encapsulation, the cryosurgery inhibited tumor growth with a slighter effect compared to the Ga/MPs combined cryosurgery group. Such a distinct comparison verified the enhanced anti-tumor effect deriving from Ga particle deformation. To further improve the therapy effect, combined chemotherapy was made by loading anti-tumor drugs with Ga/MPs and realized a better tumor elimination effect [Figure 5D iii].
These works indicated that LMs, with various forms, could be employed as synergistic platforms to exert multi-mode tumor therapy, especially to enhance cryoablation efficiency.
PROSPECTS OF LM IN CRYOBIOLOGY
LM-mediated cryosurgery
As mentioned above, cryosurgery attracts increasing attention based on the characteristics of minimally invasive and lower side effects than traditional surgical treatment. By focusing on the key points during the operation, LMs have been applied to enhance the biological heat transfer successfully, therefore achieving sufficient freezing to target tumors and showing great potency in the animal model of melanoma. It has been reported that LMs can act as an extraordinary contrast agent for X-ray visualization; thus, it is promising to realize accurate cryosurgical guidance through solid tumor vascular network injection of LMs. Moreover, LMs are also prospective in synergistic immunotherapy and direct tumor killing by iron deprivation to boost tumor treatment efficiency when combined with cryosurgery, all of which will be discussed in detail below.
LM-mediated tumor imaging optimization
Medical imaging refers to the technology of obtaining non-invasive images of internal tissues of the human body or part of the body for medical treatment or research. Since Roentgen discovered X-rays in 1895, imaging has been one of the most critical parts of clinical trials, which plays an important role in diagnosing and preoperatively planning diseases that cannot be directly observed. Tumors are highly heterogeneous with irregular shapes and are normally deeply embedded in tissues. Thus, these intrinsic properties of tumors pose a serious challenge to surgical methods, including cryosurgery, which relies on physical elimination of tumor tissues. Meanwhile, inaccurate imaging guidance can easily lead to uncontrolled cold release and cause damage to adjacent healthy tissue.
At present, most cryosurgeries are percutaneous ablation guided by CT or ultrasound, which are widely used because of their simplicity and low cost[92]. However, these two imaging methods still have shortcomings in achieving high resolution and high contrast intraoperative monitoring. With the increasing demand for precise and conformal treatment of tumor cryoablation, developing intraoperative monitoring means of high sensitivity and high resolution is of great significance in achieving accurate cryo-biomedicine.
As a multifunctional metallic material, gallium also shows a wide range of application prospects in biological imaging and can realize a multi-mode imaging ability, including CT and magnetic resonance, and it even shows powerful capability in the emerging photoacoustic imaging field[47]. The characteristics of LMs contribute greatly to imaging quality improvement. For example, LMs exhibit phenomenal X-ray absorption and are greatly radiopaque due to their higher density (6.08 g/cm3) than aqueous solutions. Gallium has been shown to be an excellent contrast agent for angiography, allowing even tiny capillaries to be visualized at high resolution due to its fluidity and compliance[93]. In tumor treatment, LMs can be injected directly into tumor tissue, while the high fluidity enables the LMs to disperse rapidly at the targeted tissue, providing complete and clear imaging of diseased tissues. In addition, LMs can also be injected through blood vessels around tumors. It is well known that tumors induce a complex blood vessel network to scramble for nutrients and oxygen. Fan et al. synthesized an injectable LM-calcium alginate hydrogel to realize local embolization, which can not only cut off the energy supply for the tumor but also act as a marker around the tumor for cryosurgery killing boundary localization[94]. Plenty of studies have demonstrated the imaging potential of LMs. However, the realization of high-quality intraoperative imaging guidance mediated by LM remains to be further developed.
Aimed at the problem of incomplete killing caused by unclear imaging of tumor tissue during cryoablation so far, efficient intraoperative imaging mediated by LMs is worthy of expectation. It is expected that LM-mediated intraoperative imaging will provide potent medical guidance for the planning and ablation of cryosurgery to achieve maximum tumor-killing efficiency and avoid tumor residuals and recurrence [Figure 6A].
Figure 6. (A) LM enhances the imaging of CT and MRI in cryosurgery; (B) LM as an immune adjuvant for synergistic immune therapy; (C) Gallium ion-mediated cancer treatment through iron deprivation; (D) Strengthening heat transfer of cryopreservation through LM coating; (E) Mechanism of anti-inflammation of Ga3+ by suppressing immune cell proliferation and promoting secretion of anti-inflammatory cytokines; (F) LM as an immunomodulating agent for relieving immune rejection. LM: Liquid metal.
LM-mediated synergistic immune therapy
Adjuvant refers to a class of substances that can specifically bind to an antigen through physical or chemical means to enhance the specific immunity of the antigen. In the past few decades, a variety of adjuvants have been developed and applied in vaccine manufacturing successfully. Among these, aluminum, as one of the most popular immune adjuvants, has been widely used in vaccine manufacturing and other fields since it was first discovered by Glenny in 1926. Therefore, it is worth considering that gallium, as a congener of aluminum, may also be an excellent immune adjuvant, as presented in Figure 6B.
Immunotherapy is a potential research direction for cancer treatment in the future[95], with the aid of tumor vaccine to release the immunosuppressive state and stimulate the patient’s own immune system so as to achieve the purpose of controlling and further eliminating the tumor. A key role of immune adjuvants in tumor vaccines is to boost the uptake and cross-presentation of antigen-presenting cells[96]. It is reported that LM nanoparticle-based nano-vaccine can enhance antigen-presenting. Meanwhile, it can induce moderate regional inflammation under infrared radiation, thus reinforcing the immune system response and immune cell recruitment[97]. The vaccine shows an evident suppression effect in mouse breast tumor models and is thought to be highly adaptive for multi-type cancer treatment. However, the underlying molecular mechanism of this study still needs to be further explored.
Compared with traditional surgical resection and chemoradiotherapy, cryoablation can retain the antigenic activity of the tumor due to its cryogenic characteristics, which is crucial for relieving the immunosuppression of middle and advanced tumors and effectively initiating the anti-tumor immune response. The delightful result is on the strength of targeted killing of tumor cells, the recognition by the immune system after tumor cells rupture, and the release of antigens mediated by cryosurgery. Studies have also shown that cryoablation can induce the abscopal effect, which refers to the reduction or even disappearance of the tumor that has metastasized to other organs and tissues in the process of tumor treatment, and this effect has been proved by cryoablation for prostate tumors treatment[98].
Hence, it is worth expecting that the synergistic immune therapeutic effect of cryoablation and LMs, along with their distinctive physical characteristics under cryogenic temperature, will bring new prospects for cancer treatment.
LM-mediated iron deprivation for tumor killing
Aluminum, gallium, and indium are three metallic elements in the periodic table with increasing atomic numbers in the same group IIIA. Thus, it is self-evident that they share certain similarities in their chemical properties [Table 3]. However, numerous in vitro and in vivo biological experiments have demonstrated that gallium and iron ions show more evident similarities in the physiological environment. This precise resemblance in biochemical properties between these two ions constitutes the basis of a series of physiological effects of gallium so that it could be widely applied to various clinical occasions, including anticancer and antibacterial practice[99].
Comparison among Ga3+, Fe3+, Al3+, and In3+
Ionic radius (octahedral) (Å) | Ionic radius (tetrahedral) (Å) | Ionization potential (eV) | Electro-negativity (eV) | Metal-oxygen bond dissociation energy (kJ·mol-1) | |
Ga3+ | 0.620 | 0.47 | 64 | 1.81 | 353.5 |
Fe3+ | 0.645 | 0.49 | 54.8 | 1.83 | 390.4 |
Al3+ | 0.535 | 0.39 | 119.99 | 1.61 | 511 |
In3+ | 0.800 | 0.62 | 54 | 1.78 | 320.1 |
The high degree of chemical similarity between gallium and ferric ions is largely due to their proximity in ionic radius and bonding [Table 3]. Therefore, in living organisms, Ga3+ can replace Fe3+ when it binds with certain proteins, such as transferrin[100], lactoferrin[101], and ferritin[102], thus further blocking the implementation of corresponding functions. It is worth noting that unlike Fe3+, which can be efficiently converted into Fe2+ or vice versa, Ga3+ does not undergo the reduction reaction under physiological conditions, which effectively prevents Ga3+ from entering the protein bounded with Fe2+ and blocks its function. Therefore, Ga3+ does not enter the blood cells and binds with hemoglobin to interfere with the oxygen transport process[99].
In practical gallium compounds related to cancer treatment, Ga3+ is just based on the aforementioned principle, which competitively binds with proteins over Fe3+, blocking the physiological process of cancer cells in the presence of specific proteins so as to achieve its anticancer role [Figure 6C]. Gallium shows a strong anti-mitotic ability of cancer cells. An important reason is that malignant tumor cells show greater iron demand than normal cells[103]; therefore, cancer cells possess numerous transferrin receptors distributed on their surface[104], facilitating the rapid transportation of gallium into cells. The competitive transport results in the conformational change and inactivation of nucleotide reductase, which prevents DNA synthesis in cancer cells and stops cancer cell mitosis at the S phase in the cell cycle, ultimately preventing the massive proliferation of cancer cells. The iron-depriving inhibition effect has been observed in several types of cancer, including human acute lymphoblastic leukemia and glioblastoma[105,106]. Furthermore, iron deprivation will induce apoptosis that enhances the suppression of cancer cell proliferation[107]. Although high concentrations of gallium ions can cause chromosomal abnormalities and nuclear damage, gallium shows low toxicity at therapeutic concentrations and can only be enriched in specific cells with high transferrin receptors.
The direct therapeutic effects in cancer treatment of gallium endow LMs with great potential in cancer treatment when combined with cryosurgery. It is promising that Ga3+ will remedy incomplete tumor killing and prevent tumor recurrence after cryosurgery.
LM-mediated cryopreservation
There are few studies concentrating on the application of LMs in cryopreservation, whereas the prospect of accomplishing LM-mediated cryopreservation is intriguing. Based on one of the critical obstacles that tissues are unfavorable in thermal conductivity for achieving excellent cryopreservation, LMs are expected to accelerate the heat dissipation so as to rewarm biosamples uniformly and rapidly. The nanoscale LM has been shown to achieve a rapid heating rate in vitrification by PT stimulation. However, the possible application of bulk LMs and the combination with various external fields is worth further investigation. It is also found that LMs, specifically gallium, have anti-inflammation and immunomodulation abilities, which endow them with a great chance to alleviate freezing-induced inflammation and regulate graft rejection.
LM-mediated heat transfer enhancement
The efficient preservation of biological samples is an important advance in the history of human medicine. At present, there are relatively sufficient studies and mature techniques for the preservation of cells and small-scale tissues. However, for large-scale tissues and organs, there is still an unbridgeable gap regarding heat transfer between biological samples and temperature-controlling medium in the process of heating and cooling procedures. It is a practical method to enhance heat exchange through by modifying characteristics, which has been applied for heat dissipation of electronic devices. The modified interface between biospecimens and the outer solution will enhance the energy transport process directly. In addition, researchers have also demonstrated that a variety of metal materials, such as metal foil, foam, and mesh, can achieve an impressive rapid warming rate of more than 1,000 °C/min due to the remarkable induced eddy currents under the radiofrequency field that generate heating through concurrent resistive losses[26].
For the purpose of conformally enhancing heat transfer and achieving a rapid warming rate, LMs are superior to any other metals that had been tested in previous work, including copper and aluminum, because of their excellent intrinsic flexibility and shape adaptability. They can be quickly coated on the surface of biological samples before cryopreservation, and samples with irregular shapes can also be well coated without dead spots, which avoids complex metal layer preparation and preservation defects caused by imperfect metal coverage [Figure 6D].
LM-mediated anti-freezing-induced inflammation
Cryopreservation achieves long-term preservation by lowering the temperature to reduce cell metabolism. However, low temperature is also a major cause of damage to biological samples, such as organs, when preserved by hypothermic storage or hypothermic machine perfusion. At the macro level, possible organ damage includes the destruction of vascular networks and tissue structure, which is closely related to mechanical damage of ice crystals and thermal stress during cooling and rewarming[108]. At the micro-level, the blocked blood flow for organs will trigger ischemic injury in the process of preservation. Long-term ischemia will lead to hypoxia, metabolic disorders, calcium overload, and a cascade of other problems. In addition, mitochondria are the main target of hypothermia damage, which causes rapid adenosine triphosphate (ATP) consumption and enzyme activity decrease; it is reported that around 95% ATP will be consumed in the first 4h when stored in 0~4 °C[109], which is harmful to the ATP-based ion exchange (e.g., Na+/K+-ATPase) and damage the cytoskeleton and membrane integrity, and then, accumulated metabolic and ion imbalances will pose a serious threat to cell survival[110]. Low temperature also gives rise to the loss of cellular antioxidant capacity. Subsequently, the unbalanced antioxidant defense system induces oxidative stress in cells, producing large amounts of reactive oxygen species (ROS) and ultimately leading to cell necrosis and apoptosis[111]. It is also found that gene transcription and protein synthesis are also affected by the expressions of apoptosis-sensitive genes, such as Bcl-2/Bax, and the activation of caspase series proteases with respect to cell apoptosis[112].
Meanwhile, the apoptotic and necrotic cells will induce the release of damage-associated molecular patterns (DAMPs)[113]. As a result of the combined adverse conditions above, organs are prone to suffer inflammation during and after preservation. Generally speaking, inflammation is an active protective response produced by the body in the face of pathogens, tissue damage, and other adverse conditions. A range of immune cells, including T cells and macrophages, are involved in the inflammatory response and synthesis and release of cytokines, which mediate a series of processes targeting immune stimulation. However, prolonged inflammation is extremely unfavorable and can easily lead to tissue dysfunction, cancer, necrosis, and even death. Ga3+ has shown great potential in immunomodulation to suppress the reaction of the immune system without evident cytotoxicity. Makkonen et al. proved that Ga3+ can suppress the impact of macrophages on immune response by inhibiting the production of inflammatory mediators[114]. Inversely, gallium could upregulate anti-inflammatory cytokines, such as IL-10, which is of great significance in inflammation resolution [Figure 6E][115]. Besides, the function of lymphocytes is also modulated by transferrin-gallium complexes that restrain the lymphocyte proliferation and immunoglobulin production greatly[116]. Chang et al. demonstrated that with a certain concentration of gallium treatment (1-10 µg/ml), peripheral blood mononuclear cells would be arrested in the S phase of the cell cycle[117].
Iron is one of the most important elements for almost all cells because of its indispensable role in a large number of Fe-dependent enzymes and proteins. Its significance lies in its essential contribution to cell survival, proliferation, and differentiation. Iron deficiency can induce anemia, while excessive iron is responsible for ferroptosis due to the overproduction of superoxide and hydroxyl radicals. Thus, the iron homeostasis is a delicate balance between import, storage, utilization, and export[103,118]. As mentioned above, the application of Ga3+ may interfere with the Fe-dependent physiological function and trigger unexpected negative effects. However, Zhang et al. discovered a unique anti-inflammatory pathway by gallium nanodroplets (GNDs) independent of Ga3+-Fe3+ competitive binding. Different from the Ga3+ nonspecific anti-inflammatory mediator inhibition, which is harmful to the cellular iron homeostasis, GNDs can upregulate eIF2α phosphorylation. This specific action disturbs the synthesis of inducible nitric oxide so that none of the activated macrophages showed any sign of iron homeostasis interference[119].
LM-mediated graft rejection relief
Organ transplantation is a historic progress in human medicine and health development, and it is the only effective method for the treatment of a great deal of end-stage diseases. Nowadays, the demand for organ transplantation is still unmet. Cryopreservation is acknowledged as a way to significantly extend the preservation time of isolated organs, which greatly alleviates the current contradiction between organ supply and demand. Beyond that, however, organ transplantation remains confronted with grim challenges. Many recipients are tortured by the serious immune rejection after receiving the transplanted organ, resulting in dysfunction of the graft, and acute immune reaction is even life-threatening. Therefore, immunosuppressants were introduced to alleviate the immune responses, which effectively improved the transplant prognosis[120].
As mentioned above, gallium is thought to be an immunomodulating agent in relieving inflammatory responses, particularly the immune process mediated by T cells and macrophages. Its unique property intrigues researchers, prompting an investigation into whether gallium could act as a metal ion immunosuppressant to attenuate immunological rejection after transplantation. Orosz et al. applied gallium to suppress the rejection in a cardiac allograft transplantation mouse model, indicating that the survival of mice was significantly improved[121]. Meanwhile, histological tests showed mitigated immune rejection. The other independent experiment also confirmed the immunomodulating potential of gallium in transplantation[122].
Therefore, it is reasonable to further speculate that gallium can be applied as a unique metallic substance added to CPAs before cryopreservation. When the organ is transplanted into the recipient, gallium at millimole levels can rapidly affect the immune system, avoiding both violent inflammatory responses and grievous immune rejection, which are fundamentally dependent on the immunomodulatory effects of gallium on T cells and macrophages [Figure 6F]. In addition, a substantial body of literature has also claimed that gallium is a potent antibacterial substance compared with traditional antibiotics in that pathogens have already become highly resistant to abused antibiotics, such as penicillin, whereas no drug resistance is observed after gallium treatment. The antibacterial effect of gallium relies on the disturbance to the normal physiological activity of bacteria, producing a great amount of ROS and further affecting microbial DNA and protein synthesis[123,124]. Truong et al. systemically summarized the fundamental mechanisms of the antimicrobial capability of gallium nanoparticles and gallium Ga3+, which are as follows: (1) membrane disruption caused by Ga3+-induced oxidative stress; (2) Iron deprivation caused by Ga3+-Fe3+ competitive binding; (3) Ga3+ binds with bacterial DNA and further causes DNA fragmentation; (4) Ga3+ binds with bacterial proteins and disrupts the bacterial metabolism[125]. Compared with solid metal antimicrobial agents, Ga-based LMs are easy to synthesize from bulk to multi-dimension particles, showing remarkable convenience in matching bacteria. It is self-evident that gallium has already become one of the most promising antibacterial agents, with the potential for more prosperous future applications.
The emerging LM-mediated cryo-biomedicine is an interdisciplinary field with the efforts and cooperation of material science, cryogenic engineering, and biomedicine. It is necessary to realize that LM-mediated cryo-biomedicine is still in its initial stage, presenting a landscape where challenges and opportunities coexist. Further development still requires efforts in underlying mechanisms, material design and synthesis, and multi-scale regulation. A few issues still ask for further explorations: (1) LM-mediated synergistic effect is worth pursuing. The combination of LMs and cryo-biomedicine provides a new approach in the face of challenges. Researchers should explore more potential applications of LMs and their composites; (2) Explore the application of LMs at nanoscales. Recently, the LM nanoparticles have been attracting increasing attention compared to their bulk counterpart due to their unique properties in heat and mass transfer. Thus, a more comprehensive understanding of LM nanoparticles in cryo-biomedical systems should be considered; (3) Achieving more precise regulation is desirable by improving LM preparation programs and advanced modulating techniques; (4) The interface characteristics are of great significance for LMs. Thus, tuning the proper interface between LMs and biological systems is essential for their performance. Overall, the new rising direction holds broad prospects but also requires a joint effort from interdisciplinary contribution. We do believe that LMs will accelerate the development of cryo-biomedicine, and many breakthroughs will emerge with their promotion.
DECLARATIONS
Authors’ contributions
Proposed original conceptualization: Lu C, Yang F, Rao W
Outlined the manuscript structure: Lu C
Investigated literature and wrote the original manuscript: Lu C, Yang F
Designed original figures: Yang F
Reviewed and revised the manuscript: Lu C, Rao W
Supervised the manuscript: Rao W
Availability of data and materials
Not applicable.
Financial support and sponsorship
This work is financially supported by the National Natural Science Foundation of China (No. 51890893).
Conflicts of interest
All authors declared that there are no conflicts of interest.
Ethical approval and consent to participate
Not applicable.
Consent for publication
Not applicable.
Copyright
© The Author(s) 2024.
REFERENCES
1. Yan JF, Liu J. Nanocryosurgery and its mechanisms for enhancing freezing efficiency of tumor tissues. Nanomedicine 2008;4:79-87.
2. Hou Y, Sun Z, Rao W, Liu J. Nanoparticle-mediated cryosurgery for tumor therapy. Nanomedicine 2018;14:493-506.
3. Yuan F, Zhao G, Panhwar F. Enhanced killing of HepG2 during cryosurgery with Fe3O4-nanoparticle improved intracellular ice formation and cell dehydration. Oncotarget 2017;8:92561-77.
4. Khademi R, Mohebbi-kalhori D, Razminia A. Thermal analysis of a tumorous vascular tissue during pulsed-cryosurgery and nano-hyperthermia therapy: finite element approach. Int J Heat Mass Tran 2019;137:1001-13.
5. Liu Y, Bhattarai P, Dai Z, Chen X. Photothermal therapy and photoacoustic imaging via nanotheranostics in fighting cancer. Chem Soc Rev 2019;48:2053-108.
6. Pham L, Dahiya R, Rubinsky B. An in vivo study of antifreeze protein adjuvant cryosurgery. Cryobiology 1999;38:169-75.
7. Muldrew K, Rewcastle J, Donnelly BJ, et al. Flounder antifreeze peptides increase the efficacy of cryosurgery. Cryobiology 2001;42:182-9.
8. Jiang J, Goel R, Schmechel S, Vercellotti G, Forster C, Bischof J. Pre-conditioning cryosurgery: cellular and molecular mechanisms and dynamics of TNF-α enhanced cryotherapy in an in vivo prostate cancer model system. Cryobiology 2010;61:280-8.
9. Wang CL, Teo KY, Han B. An amino acidic adjuvant to augment cryoinjury of MCF-7 breast cancer cells. Cryobiology 2008;57:52-9.
10. Di DR, He ZZ, Sun ZQ, Liu J. A new nano-cryosurgical modality for tumor treatment using biodegradable MgO nanoparticles. Nanomedicine 2012;8:1233-41.
11. Krishnamoorthy K, Moon JY, Hyun HB, Cho SK, Kim SJ. Mechanistic investigation on the toxicity of MgO nanoparticles toward cancer cells. J Mater Chem 2012;22:24610-7.
12. Ye P, Kong Y, Chen X, et al. Fe3O4 nanoparticles and cryoablation enhance ice crystal formation to improve the efficiency of killing breast cancer cells. Oncotarget 2017;8:11389-99.
13. Yu TH, Liu J, Zhou YX. Selective freezing of target biological tissues after injection of solutions with specific thermal properties. Cryobiology 2005;50:174-82.
14. Choi B, Choi H, Yu B, Kim DH. Synergistic local combination of radiation and anti-programmed death ligand 1 immunotherapy using radiation-responsive splintery metallic nanocarriers. ACS Nano 2020;14:13115-26.
15. Nel AE, Mädler L, Velegol D, et al. Understanding biophysicochemical interactions at the nano-bio interface. Nat Mater 2009;8:543-57.
16. Giwa S, Lewis JK, Alvarez L, et al. The promise of organ and tissue preservation to transform medicine. Nat Biotechnol 2017;35:530-42.
17. Pal R, Mamidi MK, Das AK, Bhonde R. Diverse effects of dimethyl sulfoxide (DMSO) on the differentiation potential of human embryonic stem cells. Arch Toxicol 2012;86:651-61.
18. Fahy GM, Wowk B, Wu J, et al. Cryopreservation of organs by vitrification: perspectives and recent advances. Cryobiology 2004;48:157-78.
19. Finger EB, Bischof JC. Cryopreservation by vitrification: a promising approach for transplant organ banking. Curr Opin Organ Tran 2018;23:353-60.
20. Morris GJ, Goodrich M, Acton E, Fonseca F. The high viscosity encountered during freezing in glycerol solutions: effects on cryopreservation. Cryobiology 2006;52:323-34.
21. Luyet B. On the possible biological significance of some physical changes encountered in the cooling and the rewarming of aqueous solutions. In: Cellular Injury and Resistance in Freezing Organisms: proceedings. 1967;2:1-20. Available from: http://hdl.handle.net/2115/20405. [Last accessed on 30 Nov 2023]
22. Debenedetti PG, Stillinger FH. Supercooled liquids and the glass transition. Nature 2001;410:259-67.
23. Han Z, Sharma A, Gao Z, et al. Diffusion limited cryopreservation of tissue with radiofrequency heated metal forms. Adv Healthc Mater 2020;9:2000796.
24. Manuchehrabadi N, Gao Z, Zhang JJ, et al. Improved tissue cryopreservation using inductive heating of magnetic nanoparticles. Sci Transl Med 2017;9:eaah4586.
25. Khosla K, Wang Y, Hagedorn M, Qin Z, Bischof J. Gold nanorod induced warming of embryos from the cryogenic state enhances viability. ACS Nano 2017;11:7869-78.
26. Manuchehrabadi N, Shi M, Roy P, et al. Ultrarapid inductive rewarming of vitrified biomaterials with thin metal forms. Ann Biomed Eng 2018;46:1857-69.
27. Clarkson TW, Magos L. The toxicology of mercury and its chemical compounds. Crit Rev Toxicol 2006;36:609-62.
28. Rao W, Liu J. Tumor thermal ablation therapy using alkali metals as powerful self-heating seeds. Minim Invasive Ther Allied Technol 2008;17:43-9.
29. Rao W, Liu J. Injectable liquid alkali alloy based-tumor thermal ablation therapy. Minim Invasive Ther Allied Technol 2009;18:30-5.
30. Daeneke T, Khoshmanesh K, Mahmood N, et al. Liquid metals: fundamentals and applications in chemistry. Chem Soc Rev 2018;47:4073-111.
31. Jia X, Liu B, Li S, et al. High-performance non-silicone thermal interface materials based on tunable size and polymorphic liquid metal inclusions. J Mater Sci 2022;57:11026-45.
32. Li J, Ma Q, Gao S, et al. Liquid bridge: liquid metal bridging spherical BN largely enhances the thermal conductivity and mechanical properties of thermal interface materials. J Mater Chem C 2022;10:6736-43.
33. Zhang XD, Zhang ZT, Wang HZ, Cao BY. Thermal interface materials with high thermal conductivity and low young’s modulus using a solid-liquid metal codoping strategy. ACS Appl Mater Interfaces 2023;15:3534-42.
34. Wang D, Ye J, Bai Y, et al. Liquid metal combinatorics toward materials discovery. Adv Mater 2023;35:2303533.
35. Tang J, Lambie S, Meftahi N, et al. Unique surface patterns emerging during solidification of liquid metal alloys. Nat Nanotechnol 2021;16:431-9.
36. Idrus-Saidi SA, Tang J, Lambie S, et al. Liquid metal synthesis solvents for metallic crystals. Science 2022;378:1118-24.
37. Kang M, Saucer TW, Warren MV, et al. Surface plasmon resonances of Ga nanoparticle arrays. Appl Phys Lett 2012;101:081905.
38. Qi Y, Jin T, Yuan K, You J, Shen C, Xie K. Chemically stable polypyrrole-modified liquid metal nanoparticles with the promising photothermal conversion capability. J Mater Sci Technol 2022;127:144-52.
39. Hu JJ, Liu MD, Gao F, et al. Photo-controlled liquid metal nanoparticle-enzyme for starvation/photothermal therapy of tumor by win-win cooperation. Biomaterials 2019;217:119303.
40. Sun X, Sun M, Liu M, et al. Shape tunable gallium nanorods mediated tumor enhanced ablation through near-infrared photothermal therapy. Nanoscale 2019;11:2655-67.
41. Liu T, Song Y, Huang Z, et al. Photothermal photodynamic therapy and enhanced radiotherapy of targeting copolymer-coated liquid metal nanoparticles on liver cancer. Colloid Surface B 2021;207:112023.
42. Ding XL, Liu MD, Cheng Q, et al. Multifunctional liquid metal-based nanoparticles with glycolysis and mitochondrial metabolism inhibition for tumor photothermal therapy. Biomaterials 2022;281:121369.
43. Wang D, Rao W. Alginate sponge assisted instantize liquid metal nanocomposite for photothermo-chemotherapy. Appl Mater Today 2022;29:101583.
45. Delmas T, Piraux H, Couffin AC, et al. How to prepare and stabilize very small nanoemulsions. Langmuir 2011;27:1683-92.
46. Yamaguchi A, Mashima Y, Iyoda T. Reversible size control of liquid-metal nanoparticles under ultrasonication. Angew Chem Int Ed Engl 2015;54:12809-13.
47. Chechetka SA, Yu Y, Zhen X, Pramanik M, Pu K, Miyako E. Light-driven liquid metal nanotransformers for biomedical theranostics. Nat Commun 2017;8:15432.
48. Gan T, Shang W, Handschuh-Wang S, Zhou X. Light-induced shape morphing of liquid metal nanodroplets enabled by polydopamine coating. Small 2019;15:1804838.
49. Chang H, Guo R, Sun Z, et al. Direct writing and repairable paper flexible electronics using nickel-liquid metal ink. Adv Mater Interfaces 2018;5:1800571.
50. Tang J, Zhao X, Li J, Zhou Y, Liu J. Liquid metal phagocytosis: intermetallic wetting induced particle internalization. Adv Sci 2017;4:1700024.
51. Tang J, Zhao X, Li J, Guo R, Zhou Y, Liu J. Gallium-based liquid metal amalgams: transitional-state metallic mixtures (TransM2ixes) with enhanced and tunable electrical, thermal, and mechanical properties. ACS Appl Mater Interfaces 2017;9:35977-87.
52. Zhao R, Guo R, Xu X, Liu J. A fast and cost-effective transfer printing of liquid metal inks for three-dimensional wiring in flexible electronics. ACS Appl Mater Interfaces 2020;12:36723-30.
53. Hou Y, Zhang P, Wang D, Liu J, Rao W. Liquid metal hybrid platform-mediated ice-fire dual noninvasive conformable melanoma therapy. ACS Appl Mater Interfaces 2020;12:27984-93.
54. Park YG, Min H, Kim H, Zhexembekova A, Lee CY, Park JU. Three-dimensional, high-resolution printing of carbon nanotube/liquid metal composites with mechanical and electrical reinforcement. Nano Lett 2019;19:4866-72.
55. Chang H, Zhang P, Guo R, et al. Recoverable liquid metal paste with reversible rheological characteristic for electronics printing. ACS Appl Mater Interfaces 2020;12:14125-35.
56. Wang X, Yao W, Guo R, et al. Soft and moldable mg-doped liquid metal for conformable skin tumor photothermal therapy. Adv Healthc Mater 2018;7:1800318.
57. Wang D, Wu Q, Guo R, Lu C, Niu M, Rao W. Magnetic liquid metal loaded nano-in-micro spheres as fully flexible theranostic agents for SMART embolization. Nanoscale 2021;13:8817-36.
59. Kong W, Wang Z, Wang M, et al. Oxide-mediated formation of chemically stable tungsten-liquid metal mixtures for enhanced thermal interfaces. Adv Mater 2019;31:1904309.
60. Kim H, Lee K, Oh JW, et al. Shape-deformable and locomotive MXene (Ti3C2Tx)-encapsulated magnetic liquid metal for 3D-motion-adaptive synapses. Adv Funct Mater 2023;33:2210385.
61. Murphy CJ, Sau TK, Gole AM, et al. Anisotropic metal nanoparticles: synthesis, assembly, and optical applications. J Phys Chem B 2005;109:13857-70.
62. Lin Y, Liu Y, Genzer J, Dickey MD. Shape-transformable liquid metal nanoparticles in aqueous solution. Chem Sci 2017;8:3832-7.
63. Li Z, Zhang H, Wang D, et al. Reconfigurable assembly of active liquid metal colloidal cluster. Angew Chem Int Ed Engl 2020;59:19884-8.
64. Wang D, Gao C, Wang W, et al. Shape-transformable, fusible rodlike swimming liquid metal nanomachine. ACS Nano 2018;12:10212-20.
65. Sun X, Guo R, Yuan B, et al. Low-temperature triggered shape transformation of liquid metal microdroplets. ACS Appl Mater Interfaces 2020;12:38386-96.
66. Hou Y, Qiao Y, Zhang D, et al. Numerical simulation for treatment of hypothermia based on vascular interventional direct heating system. J Therm Biol 2018;76:29-37.
67. Deng Y, Liu J. Hybrid liquid metal-water cooling system for heat dissipation of high power density microdevices. Heat Mass Transfer 2010;46:1327-34.
68. Luo M, Liu J. Experimental investigation of liquid metal alloy based mini-channel heat exchanger for high power electronic devices. Front Energy 2013;7:479-86.
69. Wang L, Zhang XD, Liu J, Zhou YX. Heat dissipation system based on electromagnetic-driven rotational flow of liquid metal coolant. J Thermal Sci Eng Appl 2021;13:061023.
70. Deng Y, Zhang M, Jiang Y, Liu J. Two-stage multichannel liquid-metal cooling system for thermal management of high-heat-flux-density chip array. Energy Convers Manag 2022;259:115591.
71. Gao Y, Liu J. Gallium-based thermal interface material with high compliance and wettability. Appl Phys A 2012;107:701-8.
72. Wang X, Fan L, Zhang J, et al. Printed conformable liquid metal e-skin-enabled spatiotemporally controlled bioelectromagnetics for wireless multisite tumor therapy. Adv Funct Mater 2019;29:1907063.
73. Fan P, Sun Z, Wang Y, et al. Nano liquid metal for the preparation of a thermally conductive and electrically insulating material with high stability. RSC Adv 2018;8:16232-42.
74. Panhwar F, Chen Z, Hossain SMC, et al. Near-infrared laser mediated modulation of ice crystallization by two-dimensional nanosheets enables high-survival recovery of biological cells from cryogenic temperatures. Nanoscale 2018;10:11760-74.
75. Cao Y, Chang T, Fang C, Zhang Y, Liu H, Zhao G. Inhibition effect of Ti3C2Tx MXene on ice crystals combined with laser-mediated heating facilitates high-performance cryopreservation. ACS Nano 2022;16:8837-50.
76. Zhan T, Liu K, Yang J, Dang H, Chen L, Xu Y. Fe3O4 nanoparticles with carboxylic acid functionality for improving the structural integrity of whole vitrified rat kidneys. ACS Appl Nano Mater 2021;4:13552-61.
77. Tian C, Shen L, Gong C, Cao Y, Shi Q, Zhao G. Microencapsulation and nanowarming enables vitrification cryopreservation of mouse preantral follicles. Nat Commun 2022;13:7515.
78. Jain PK, Huang X, El-Sayed IH, El-Sayed MA. Noble metals on the nanoscale: optical and photothermal properties and some applications in imaging, sensing, biology, and medicine. Acc Chem Res 2008;41:1578-86.
79. Hu JJ, Liu MD, Chen Y, et al. Immobilized liquid metal nanoparticles with improved stability and photothermal performance for combinational therapy of tumor. Biomaterials 2019;207:76-88.
81. Han Z, Rao JS, Gangwar L, et al. Vitrification and nanowarming enable long-term organ cryopreservation and life-sustaining kidney transplantation in a rat model. Nat Commun 2023;14:3407.
82. Wang D, Xie W, Gao Q, et al. Non-magnetic injectable implant for magnetic field-driven thermochemotherapy and dual stimuli-responsive drug delivery: transformable liquid metal hybrid platform for cancer theranostics. Small 2019;15:1900511.
83. Kim JH, Kim S, So JH, Kim K, Koo HJ. Cytotoxicity of gallium-indium liquid metal in an aqueous environment. ACS Appl Mater Interfaces 2018;10:17448-54.
84. Guo R, Liu J. Implantable liquid metal-based flexible neural microelectrode array and its application in recovering animal locomotion functions. J Micromech Microeng 2017;27:104002.
85. Sun X, Cui B, Yuan B, et al. Liquid metal microparticles phase change medicated mechanical destruction for enhanced tumor cryoablation and dual-mode imaging. Adv Funct Materials 2020;30:2003359.
86. Yan J, Zhang X, Liu Y, et al. Shape-controlled synthesis of liquid metal nanodroplets for photothermal therapy. Nano Res 2019;12:1313-20.
87. Hou Y, Lu C, Dou M, et al. Soft liquid metal nanoparticles achieve reduced crystal nucleation and ultrarapid rewarming for human bone marrow stromal cell and blood vessel cryopreservation. Acta Biomater 2020;102:403-15.
88. Zhang C, Tang J, Xie W, et al. Mechanistic observation of interactions between macrophages and inorganic particles with different densities. Small 2023;19:2204781.
89. Yang N, Li W, Gong F, et al. Injectable nonmagnetic liquid metal for eddy-thermal ablation of tumors under alternating magnetic field. Small Methods 2020;4:2000147.
90. Galvao J, Davis B, Tilley M, Normando E, Duchen MR, Cordeiro MF. Unexpected low-dose toxicity of the universal solvent DMSO. FASEB J 2014;28:1317-30.
91. Wang X, Li X, Duan M, et al. Endosomal escapable cryo-treatment-driven membrane-encapsulated Ga liquid-metal transformer to facilitate intracellular therapy. Matter 2022;5:219-36.
92. Zhang X, Tian J, Zhao L, et al. CT-guided conformal cryoablation for peripheral NSCLC: initial experience. Eur J Radiol 2012;81:3354-62.
93. Wang Q, Yu Y, Pan K, Liu J. Liquid metal angiography for mega contrast X-ray visualization of vascular network in reconstructing in-vitro organ anatomy. IEEE Trans Biomed Eng 2014;61:2161-6.
94. Fan L, Duan M, Xie Z, et al. Injectable and radiopaque liquid metal/calcium alginate hydrogels for endovascular embolization and tumor embolotherapy. Small 2020;16:1903421.
95. Pardoll DM. The blockade of immune checkpoints in cancer immunotherapy. Nat Rev Cancer 2012;12:252-64.
96. Mellman I, Steinman RM. Dendritic cells: specialized and regulated antigen processing machines. Cell 2001;106:255-8.
97. Zhang Y, Liu MD, Li CX, Li B, Zhang XZ. Tumor cell membrane-coated liquid metal nanovaccine for tumor prevention. Chin J Chem 2020;38:595-600.
98. Yakkala C, Chiang CLL, Kandalaft L, Denys A, Duran R. Cryoablation and immunotherapy: an enthralling synergy to confront the tumors. Front Immunol 2019;10:2283.
99. Bernstein LR. Mechanisms of therapeutic activity for gallium. Pharmacol Rev 1998;50:665-82. Available from: https://pharmrev.aspetjournals.org/content/50/4/665. [Last accessed on 30 Nov 2023].
100. Clausen J, Edeling CJ, Fogh J. 67Ga binding to human-serum proteins and tumor components. Cancer Res 1974;34:1931-7. Available from: https://aacrjournals.org/cancerres/article/34/8/1931/480195. [Last accessed on 30 Nov 2023].
101. Harris WR. Thermodynamics of gallium complexation by human lactoferrin. Biochemistry 1986;25:803-8.
102. Weiner RE. Role of phosphate-containing compounds in the transfer of indium-111 and gallium-67 from transferrin to ferritin. J Nucl Med 1989;30:70-9. Available from: https://jnm.snmjournals.org/content/30/1/70. [Last accessed on 30 Nov 2023].
103. Chitambar CR. Gallium and its competing roles with iron in biological systems. Biochim Biophys Acta 2016;1863:2044-53.
104. Chitambar CR, Seligman PA. Effects of different transferrin forms on transferrin receptor expression, iron uptake, and cellular proliferation of human leukemic HL60 cells. Mechanisms responsible for the specific cytotoxicity of transferrin-gallium. J Clin Invest 1986;78:1538-46.
105. Hedley DW, Tripp EH, Slowiaczek P, Mann GJ. Effect of gallium on DNA-synthesis by human T-cell lymphoblasts. Cancer Res 1988;48:3014-8. Available from: https://aacrjournals.org/cancerres/article/48/11/3014/492615. [Last accessed on 30 Nov 2023].
106. Chitambar CR, Al-Gizawiy MM, Alhajala HS, et al. Gallium maltolate disrupts tumor iron metabolism and retards the growth of glioblastoma by inhibiting mitochondrial function and ribonucleotide reductase. Mol Cancer Ther 2018;17:1240-50.
107. Chitambar CR, Purpi DP, Woodliff J, Yang M, Wereley JP. Development of gallium compounds for treatment of lymphoma: gallium maltolate, a novel hydroxypyrone gallium compound, induces apoptosis and circumvents lymphoma cell resistance to gallium nitrate. J Pharmacol Exp Ther 2007;322:1228-36.
108. Feng H, Xu Y, Luo S, Dang H, Liu K, Sun WQ. Evaluation and preservation of vascular architectures in decellularized whole rat kidneys. Cryobiology 2020;95:72-9.
109. Maathuis MHJ, Leuvenink HGD, Ploeg RJ. Perspectives in organ preservation. Transplantation 2007;83:1289-98.
110. Ma Y, Gao L, Tian Y, Chen P, Yang J, Zhang L. Advanced biomaterials in cell preservation: hypothermic preservation and cryopreservation. Acta Biomater 2021;131:97-116.
111. Niu X, Arthur PG, Jeffrey GP. Iron and oxidative stress in cold-initiated necrotic death of rat hepatocyte. Transplant Proc 2010;42:1563-8.
112. Zhang TJ, Hang J, Wen DX, Hang YN, Sieber FE. Hippocampus bcl-2 and bax expression and neuronal apoptosis after moderate hypothermic cardiopulmonary bypass in rats. Anesth Analg 2006;102:1018-25.
113. Zhai Y, Petrowsky H, Hong JC, Busuttil RW, Kupiec-Weglinski JW. Ischaemia-reperfusion injury in liver transplantation - from bench to bedside. Nat Rev Gastroenterol Hepatol 2013;10:79-89.
114. Makkonen N, Hirvonen MR, Savolainen K, Lapinjoki S, Mönkkönen J. The effect of free gallium and gallium in liposomes on cytokine and nitric oxide secretion from macrophage-like cells in vitro. Inflamm Res 1995;44:523-8.
115. de Albuquerque Wanderley Sales V, Timóteo TRR, da Silva NM, et al. A systematic review of the anti-inflammatory effects of gallium compounds. Curr Med Chem 2021;28:2062-76.
116. Chitambar CR, Seigneuret MC, Matthaeus WG, Lum LG. Modulation of lymphocyte proliferation and immunoglobulin production by transferrin-gallium. Cancer Res 1989;49:1125-29. Available from: https://aacrjournals.org/cancerres/article/49/5/1125/494569. [Last accessed on 30 Nov 2023].
117. Chang KL, Liao WT, Yu CL, Lan CCE, Chang LW, Yu HS. Effects of gallium on immune stimulation and apoptosis induction in human peripheral blood mononuclear cells. Toxicol Appl Pharmacol 2003;193:209-17.
118. Crichton RR, Wilmet S, Legssyer R, Ward RJ. Molecular and cellular mechanisms of iron homeostasis and toxicity in mammalian cells. J Inorg Biochem 2002;91:9-18.
119. Zhang C, Yang B, Biazik JM, et al. Gallium nanodroplets are anti-inflammatory without interfering with iron homeostasis. ACS Nano 2022;16:8891-903.
120. Bamoulid J, Staeck O, Halleck F, et al. The need for minimization strategies: current problems of immunosuppression. Transpl Int 2015;28:891-900.
121. Orosz CG, Wakely E, Bergese SD, et al. Prevention of murine cardiac allograft rejection with gallium nitrate. Comparison with anti-CD4 monoclonal antibody. Transplantation 1996;61:783-91.
122. Drobyski WR, Ul-haq R, Majewski D, Chitambar CR. Modulation of in vitro and in vivo T-cell responses by transferrin- gallium and gallium nitrate. Blood 1996;88:3056-64.
123. Li L, Chang H, Yong N, Li M, Hou Y, Rao W. Superior antibacterial activity of gallium based liquid metals due to Ga3+ induced intracellular ROS generation. J Mater Chem B 2021;9:85-93.
124. Divakarla SK, Das T, Chatterjee C, et al. Antimicrobial and anti-inflammatory gallium-defensin surface coatings for implantable devices. ACS Appl Mater Interfaces 2022;14:9685-96.
Cite This Article
Export citation file: BibTeX | RIS
OAE Style
Yang F, Lu C, Rao W. Liquid metals enabled advanced cryobiology: development and perspectives. Soft Sci 2024;4:9. http://dx.doi.org/10.20517/ss.2023.43
AMA Style
Yang F, Lu C, Rao W. Liquid metals enabled advanced cryobiology: development and perspectives. Soft Science. 2024; 4(1): 9. http://dx.doi.org/10.20517/ss.2023.43
Chicago/Turabian Style
Yang, Fan, Chennan Lu, Wei Rao. 2024. "Liquid metals enabled advanced cryobiology: development and perspectives" Soft Science. 4, no.1: 9. http://dx.doi.org/10.20517/ss.2023.43
ACS Style
Yang, F.; Lu C.; Rao W. Liquid metals enabled advanced cryobiology: development and perspectives. Soft. Sci. 2024, 4, 9. http://dx.doi.org/10.20517/ss.2023.43
About This Article
Special Issue
Copyright
Data & Comments
Data
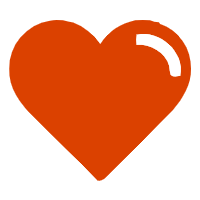

Comments
Comments must be written in English. Spam, offensive content, impersonation, and private information will not be permitted. If any comment is reported and identified as inappropriate content by OAE staff, the comment will be removed without notice. If you have any queries or need any help, please contact us at support@oaepublish.com.